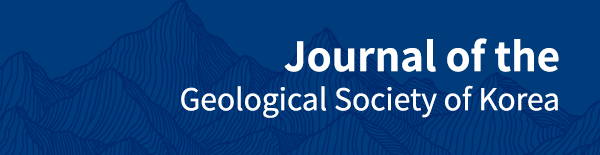
KURT 부지 암반 초기응력 특성 규명
Copyright © 2017 The Geological Society of Korea
This is an Open Access article distributed under the terms of the Creative Commons Attribution Non-Commercial License (http://creativecommons.org/licenses/by-nc/3.0/ which permits unrestricted non-commercial use, distribution, and reproduction in any medium, provided the original work is properly cited.
초록
KURT (Korea Atomic Energy Research Institute Underground Research Tunnel) 부지 내 굴착된 시추공에서 수압파쇄시험 및 공내 영상검층을 수행하여 연구 지역 암반의 초기응력 상태를 분석하였다. 일련의 시추공 현장 시험 결과에서 관찰한 수압파쇄 인장균열 및 시추공 공벽파괴의 방향성은 연구 지역에 동서 방향의 최대 수평주응력(SHmax)이 작용하고 있음을 지시한다. 최소수평주응력(Shmin)의 크기는 수압파쇄시험에서 얻어진 시간-압력 곡선을 분석해 규명하였고, SHmax의 크기는 영상검층에서 관찰된 공벽파괴와 암반 물성의 관계를 이용해 추정하였다. 연구 지역에서 상재하중으로 계산한 연직응력(Sv)은 깊이에 따라 최소주응력에서 중간주응력으로 변하는 경향을 보이며, 이는 KURT 부지가 역단층(Sv < Shmin < SHmax) 또는 주향이동단층(Shmin < Sv < SHmax) 운동에 유리한 응력 체계(stress regime) 하에 있음을 지시한다. 본 연구의 응력 측정 결과는 기존에 연구된 한반도 지역 지각 응력 특성들과 일치한다.
Abstract
We conducted hydraulic fracturing (HF) tests and borehole image logging, to investigate in situ stress state at KURT (Korea Atomic Energy Research Institute Underground Research Tunnel) site, Daejeon, Korea. The HF tensile fractures and drilling induced borehole wall failures, observed from borehole images, consistently indicated the E-W orienting maximum horizontal principal stress (SHmax). The magnitudes of the minimum horizontal principal stress (Shmin) were determined using the pressure-time curves recorded during HF tests and those of SHmax using the relationship between logged borehole wall failure and rock strength. The vertical stress (Sv), calculated from overburden weight of rocks, was either the minimum or the intermediate principal stress depending on depths. It indicates that the stress regime in KURT site is variable between thrust faulting (Sv < Shmin < SHmax) and strike-slip faulting (Shmin < Sv < SHmax) regimes. Our stress estimation results agree with the regional scale crustal stress pattern in Korean Peninsula reported from the previous studies.
Keywords:
KURT, In situ stress, Hydraulic fracturing, Borehole wall failures키워드:
KURT, 초기응력, 수압파쇄시험, 시추공 공벽파괴1. 서 론
최근 잇따른 지진 발생에 따라 원자력 에너지의 안전한 이용, 탈 원전 정책 등이 이슈화되고 있으며 이와 더불어 고준위방사성폐기물의 안전한 처분에 대해서도 관심이 높아지고 있다. 고준위방사성폐기물의 처분과 관련하여, 한국원자력연구원 부지 내 지하연구시설(Korea Atomic Energy Research Institute Underground Research Tunnel, KURT)을 이용하여 심지층처분시스템을 개발하고, 부지 암반의 심부 지질 환경을 특성화하는 다양한 연구들이 진행 중이다(Kwon and Cho, 2007; Cho et al., 2008; Kwon et al., 2011; Lee et al., 2011; Ji et al., 2013; Yoon et al., 2013).
심부 암반의 지질학적, 암반역학적 특성 중 하나인 원위치 초기응력(in situ stress) 상태는 지하시설 건설 및 터널 굴착에 의한 공동 주변의 응력 분포를 예상하고, 터널 벽면 암석 돌출(rock burst) 등 잠재적 불안정 요소의 발생 가능성을 평가하는데 필요한 주요 인자이다(Martin and Lanyon, 2003). 또한 장기적으로는 암반 내에 존재하는 단층 또는 균열의 전단 활성 및 이에 동반된 지진 발생 등 불연속면의 지질역학적 거동 특성을 예측하는데 요구되는 매개변수이며(Scholz, 2002; Zoback et al., 2003; Moeck et al., 2009), 방사성폐기물 처분, 지열 발전, 이산화탄소 지중 처분 등을 위한 장심도 시추공의 설계 및 안정성 평가에도 필요한 인자이다(Evans et al., 2005; Hawkes et al., 2005; Brady et al., 2009).
암반에 작용하는 초기응력 상태는 시추공을 이용한 수압파쇄시험, 공내 영상검층 등의 현장 시험으로 직접 측정할 수 있다(Zoback et al., 2003; Schmitt et al., 2012; Stephansson and Zang, 2012). 직접 측정하기 어려운 경우, 심부 암반의 응력 상태는 대체적으로 지구조 운동에 의해 발생하는 광역 규모(tectonic scale)의 지각 응력 전파 양상을 따르는 경향이 있기 때문에 인접 지역의 응력 분포 특성을 이용해 유추하거나, 지진 포컬메커니즘 분석(focal mechanism analysis) 등을 통해 그 경향성을 일정 정도 파악하기도 한다. 국외에서는 석유, 토목 업계를 기반으로 1980년대 이후 시추 및 수압파쇄 기술이 체계화되면서 많은 양의 응력 측정 자료가 축적되어 왔으며, 최근에는 연구 목적으로 수 km 심부에 대한 응력 측정도 수행하고있다(Zoback et al., 1993; Zoback and Brudy, 1997; Harjes, 1997). 또한, 이렇게 누적된 자료들을 취합하여 대륙 및 국가별 심부 지각 응력의 패턴을 세계응력지도(World Stress Map)로 편찬해 활용하고 있다(Heidbach et al., 2010; 2016). 반면, 국내의 경우 초기응력은 광산개발, 터널 및 토목 공사에 필요한 기초자료로써 획득되어 왔기 때문에 대부분의 측정 결과가 300 m 이내의 천부에 국한되어 있는 실정이다(Haimson et al., 2003; Bae and Jeon, 2005, 2014; Chang et al., 2010). 최근 들어 국내 이산화탄소 지중 처분와 심부지열발전 등의 연구를 위해 굴착한 장심도 시추공에서 500 m 이상의 심부 암반에 대한 응력 측정 연구가 수행된 바 있지만(Bae et al., 2016; Chang et al., 2016), 그 수가 제한적이어서 국내 심부 응력의 전반적인 경향성을 분석하기 어렵다. 또한 초기응력 상태는 지역 별로 암반 내에 존재하는 단층 또는 층서 등의 지질 경계와 지형 조건에 기인해 불균질성을 보이는 경우가 많기 때문에(Barton and Zoback, 1994; Yale, 2003; Chang et al., 2014; Chang and Jo, 2015), 결국 URL 및 지하 구조물 건설 등의 특수한 목적에서는 대상 부지의 지질 및 구조적 특징을 반영하여 원위치 초기응력성분을 정량적으로 특성화하는 것이 중요하다.
이 같은 맥락에서, 본 연구에서는 한국원자력연구원의 KURT 부지 암반에 작용하는 초기응력 상태를 규명하였다. 이를 위해 KURT 부지에 굴착된 시추공에서 수압파쇄시험 및 공내 영상검층을 수행하였으며, 얻어진 주요 결과들을 종합하였다. 또한 규명된 암반 응력 상태의 활용 방안 등을 토의하였다.
2. 연구 지역 지질 및 시추공
연구 지역인 KURT 부지는 대전광역시 유성 지역에 위치하며, 지질은 선캠브리아기 편마암류와 중생대 심성암이 주를 이루고, 곳곳에 백악기의 맥암류가 관입하고 있다. 대전 도폭은 이 지역에 대해 쥐라기의 편마상 화강암 및 동일 마그마에서 유래된 복운모 화강암으로 기재하였으며, 부지 내의 시추공에서 회수된 코어 시료들도 이와 잘 일치한다. 암반에서 관찰되는 편리들은 주변 지역에 분포하는 변성퇴적암류의 편리 방향성과 같으며, 관입맥암류의 주방향은 NS내지 N10°W로, 현장 지질조사와 시추공 물리검층으로부터 관찰된 부지 내의 주 단층 및 투수성(또는 개구성) 단열대의 방향과도 잘 일치한다(Park et al., 2010; Oh et al., 2017).
본 연구에서 KURT 부지 암반의 초기응력 상태를 규명하기 위해 이용한 시추공(KP-2, YS-04, DB-02)의 위치를 그림 1에 표시하였다. 굴착된 세 시추공 사이의 수평 이격 거리는 약 200-500 m이다. 연구 대상부지의 최대 해발고도(elevation)는 약 255 m이며, 각 공이 굴착된 위치의 고도는 100 m 내외에서 큰 차이를 보이지 않는다. 세 시험공은 공히 연직 시추공으로서, KURT 부지의 심부 지질 환경을 평가하고, 다양한 현장 시험을 통해 지하 암반의 수리적, 역학적 특성 등을 연구하기 위한 목적으로 굴착되었다. 세 공에서 회수된 시추 코어 분석으로 평가한 부지 암반의 RQD (Rock Quality Designation)는 천부 수 m 및 일부 파쇄대 구간을 제외한 전 심도에서 평균 90% 이상의 값을 보이며, 이는 부지 암반이 비교적 신선한 상태임을 지시한다.

Stress measurement site (KURT) and borehole locations. Altitudes of individual boreholes, KP-2, YS-04, DB-02 are 120, 95, 115 m, respectively.
뒤에서 더 자세히 보이겠지만, 세 시추공 중 KP-2 (굴착 심도 160 m)와 YS-04 (굴착심도 350 m)에서는 수압파쇄시험을 수행하였으며, 이를 통해 KURT 부지 암반의 천부 응력 상태를 파악하였다. DB-02공은 굴착 심도 1,000 m의 장심도 시추공으로서, 전 구간에 걸쳐 수행한 공내 영상검층 결과 중 일부 심도에서 응력지시자인 공벽파괴가 관찰되었으며, 이를 이용해 보다 깊은 심도에서의 응력 상태를 유추하고자 하였다.
3. 초기응력 방향
3.1 시추공 주변 응력 상태
시추공 굴착에 의한 공 주변의 수평응력 재배열은 공벽 및 암반에 국지적인 응력 집중으로 나타난다. 이 때 시추공 주변에서 유효한 암반 응력 상태는 Kirsch (1898) 해법에 의해 모사되며, 유효 응력 성분들은 공벽에 대해 접선방향으로 작용하는 응력(tangential stress, σθθ), 직경 방향의 응력(radial stress, σrr), 그리고 공 축과 나란한 연직방향의 응력(axial stress, σzz)으로 나타난다. 이 때, 공벽면에 작용하는 유효 응력 성분과 암반 초기응력과의 관계는 아래 식 (1) 로 표현된다(e.g., Zoback et al., 2003; Jaeger et al., 2009) (그림 2).
(1) |

Schematic of effective stress state at the borehole wall. Both tangential stress (σθθ) and axial stress (σzz) vary with azimuth (θ). Under the east-west compression state, the maximum or minimum σθθ are induced at azimuths of NS (θ=90, 270º) or EW (θ=0, 180º), respectively. The Pm is borehole annulus pressure. If both Pm and P0 are hydrostatic, the radial stress (σrr) is 0. C0 and T0 denote compressive and tensile strength of rock, respectively. When induced σθθ exceeds the rock strengths, wellbore wall failure (BO or DITF) occurs (modified from Zoback et al., 2003).
위에서 SHmax와 Shmin은 각각 최대 및 최소수평주응력, Sv는 연직응력의 크기이다. θ는 최대수평주응력(SHmax)의 압축 방향(θ=0°)으로부터 시추공벽 위치별 각거리를 나타내며, ν는 암반의 포아송비, Pm은 시추공내 압력, Δ P는 공내압과 P0의 차이다. 식 (1)로 부터 공벽면을 따라 집중되는 접선응력(σθθ)의 크기는 SHmax 방향 또는 Shmin 방향에서 최소 또는 최대가 됨을 알 수 있으며, σzz의 크기 또한 θ에 따라 순환함을 파악할 수 있다. 또한, 일반적인 나공 상태에서 Pm과 P0를 정수압으로 가정하면 ΔP, 즉 σrr은 0이 된다.
위와 같이 주어진 벽면 응력 상태에서 시추공을 이용한 수압파쇄시험 시 인장균열은 수평응력의 집중이 가장 약한 방향, 즉 최대수평주응력 방향을 따라 전파되며, 이를 통해 SHmax의 방향을 규명할 수 있다(Haimson and Cornet, 2003). 이와 더불어 유도된 σθθ의 크기가 암반의 압축강도(C0) 또는 인장강도(T0)를 초과할 때 시추공벽 압축파괴(borehole breakouts, BO) 또는 인장파괴(drilling induced tensile fractures, DITF)가 발생하는데, 이 때 공벽파괴의 방향성과 그 발달 폭(width)은 응력텐서와 연관되어 있으므로, 이를 이용하면 직접적인 응력측정 시험 없이도 초기응력의 방향 및 크기를 유추할 수 있다.
3.2 시추공 수압파쇄시험
시추공을 이용한 수압파쇄 응력측정법은 공내 시험 구간 상하부를 팽창성 패커(straddle packer)로 밀폐한 후 격리 구간에 물을 주입해 압력을 증가시켜 공벽 접선방향으로 인장응력을 유도하고, 이 응력이 암반의 인장강도를 초과할 때 발생하는 수압파쇄 균열의 방향성 및 공내 압력 등의 몇 가지 주요 인자를 이용해 암반의 초기응력텐서를 규명하는 기법이다(Haimson and Cornet, 2003) (그림 3). 본 연구를 위해 수행된 수압파쇄시험에서는 압력과 주수량을 지표에서 측정하였으며 지표에서 측정한 압력에 응력측정 심도까지의 정수압을 더하여 구간 압력(interval pressure)을 산정하였다. 앞에서 언급한대로, 본 연구의 수압파쇄시험은 KURT 부지 내 시추공 2개소(KP-2, YS-04)에서 수행되었다. 먼저 시험구간 선정을 위해 시추 코어 분석과 공내 영상검층을 수행하였다. 시추공을 관통하는 파쇄대나 균열 등의 불연속면이 없는 1 m 길이 이상의 무결암(intact rock) 구간을 선별했으며, 최종적으로 결정된 응력측정 대상 심도는 KP-2 공의 6개(50.5, 69.5, 110.5, 128.5, 136.5, 154.6 m) 및 YS-04 공의 6개(117.5, 160.5, 234, 315, 328, 343.5 m) 구간이다. 그림 4는 수압파쇄시험에서 얻어진 시험 구간의 공내 압력-시간 곡선들 중 하나를 예로 보여준다. 패커로 격리된 1 m의 시험 구간에 약 2 liter/min의 일정한 유량(flow rate)으로 물을 주입해 공내 수압을 증가시켜 공벽의 인장파괴(breakdown)를 유도하였고, 파괴 직후 물주입을 멈추고 시간에 따른 압력 감쇠와 균열 닫힘(shut in) 양상을 관찰하였다. 이후 3-5회의 재가압 시험으로 수압파쇄 균열에 대한 재개방(reopening)과 shut in을 반복하여 응력 크기 분석에 필요한 주요 공내 압력들을 얻었고, 시험을 끝낸 후 임프레션 패커(impression packer)를 이용해 해당 구간 공벽에 형성된 인장균열의 형태 및 방향을 파악하였다.

An example of interval pressure and flow rate - time records, derived from the hydraulic fracturing test at a depth of 343.5 m in a borehole YS-04. Pb and Ps denote breakdown pressure and shut in pressure, respectively. P0, a dashed line, indicates the initial pore pressure at the test interval depth.
수압파쇄시험으로 유도되는 인장균열의 기하학적 형태와 그 발생 방향은 시험 결과로부터 초기응력텐서를 분석하는데 중요한 정보를 제공한다(Baumgärtner and Zoback, 1989; Chang et al., 2014). 패커로 잘 격리된 시험 구간에서 이상적인 수압파쇄는 시추공축과 나란한 연직 방향의 인장균열 형태로 발생하며, 특히 인장균열의 방위각은 곧 최대수평주응력의 압축 방향을 지시하기 때문에 시험 후 해당 구간에 형성된 균열의 방향성을 자세히 관찰하는 것이 중요하다. 그림 5는 KP-2와 YS-04 시추공 내 응력측정 시험이 수행된 12개 구간에 대하여 임프레션 패커로 각인(imprint)된 수압파쇄 균열들의 자취를 보여준다. 모든 시험 구간에서 시추공벽에 마주보는 방향으로 연직 또는 그에 준하는 경사각의 인장균열이 관찰되었으며, 이미지를 수치화(digitize)하여 균열들의 평균 방향을 산정하였다.
3.3 공내 영상검층 및 시추공 공벽파괴
본 연구에 이용한 DB-02 공에서는 직접적인 응력측정 시험이 수행되진 않았지만, 전 구간(0-959 m)에 걸쳐 수행된 공내 영상검층 결과로부터, 일부 심도에서 응력지시자인 시추공벽 압축파괴(473-482 m)와 인장파괴(559, 581, 584, 735 m)가 관찰되었다(그림 6). 식 (1) 및 그림 2로부터 알 수 있듯이, 시추공굴착에 기인하여 발생되는 BO와 DITF는 각각 최대 수평주응력에 대해 수직 또는 나란한 방향으로 형성된다. 관찰된 공벽파괴 요소들의 방위각을 분석하고, 이를 이용해 수평응력의 방향성을 산정하였다. 시추공벽 압축파괴는 약 10 m 길이의 긴 구간에 걸쳐 연속적인 파쇄대 형태로 남북방향의 마주보는 두 방위각으로 형성되었으며, 인장파괴는 수압파쇄 균열과 비슷하게 좁은 폭의 연직 균열의 형태로 공벽면 180° 간격의 동서방향을 따라 발달한 것을 관찰할 수 있다(그림 6b).
3.4 수평응력 방향성 분석
그림 7은 KP-2, YS-04 공에 대한 수압파쇄시험으로 유도된 인장균열과 DB-02 공에서 관찰된 공벽파괴 요소들의 방위각을 이용해 KURT 부지에 작용하는 초기응력의 방향성을 규명한 결과를 보여준다. 각 시추공 굴착 위치의 고도 차에 기인한 시험 심도의 차이를 보정하기 위해 해수면 기준 깊이(elevation)를 기준하여 결과를 정리하였다. 응력측정 깊이 별로 약간의 편차를 보이긴 하지만 수압파쇄 인장균열들은 대체로 동서 내지 동남동-서북서 방향으로 형성되었음을 관찰할 수 있으며, DB-02 공에서 관찰된 공벽파괴 양상은 남북(BO zone) 또는 동서방향(DITF)으로 형성되었음을 볼 수 있다(그림 7a). 수압파쇄 균열과 BO 및 DITF의 방위각을 이용하여 종합한 부지 암반 최대수평주응력의 방향은 약 N96±11°E이며(그림 7b), 이러한 결과는 기존에 연구되어온 국내 동서방향의 SHmax 압축 방향과 잘 부합한다(Jun, 1991; Haimson et al., 2003; Synn et al., 2013).

(a) Azimuths of the hydraulic tensile fractures (HF), borehole breakouts (BO), drilling induced tensile fractures (DITF), observed in the three test holes, KP-2, YS-04 and DB-02. (b) Determining orientation of SHmax. All stress indicators, derived from boreholes, consistently argue the SHmax direction of EW compression. Investigated average direction of SHmax at KURT site is N96±11ºE.
4. 응력 크기 규명
4.1 수압파쇄 응력측정
KP-2, YS-04 공에서 수행된 일련의 시추공 수압파쇄시험으로부터 얻어진 압력-시간 곡선을 분석해 KURT 부지 천부 깊이(~250 m)에 대한 초기응력 상태를 규명하였다. 식 (1)로부터 얻어지는 시추공 벽면 유효응력 조건 아래서 수압파쇄시험으로 얻어지는 균열의 발생 압력, 폐쇄 압력, 재개방 압력 등의 몇 가지 주요 공내압들을 분석하여 초기응력의 크기를 규명할 수 있다. 수압파쇄시험 중 밀폐 구간에 물을 주입하여 공내 수압을 증가시키면 접선응력(σθθ)이 점차 감소하여 시추공벽 접선방향에 대하여 벌어지는 방향으로 인장응력(hoop stress)이 발생하며, 유도된 σθθ의 크기가 인장파괴 기준(σθθ ≤ -T0)에 도달하면 공벽이 인장 파괴된다. 이 때 수압파쇄를 발생시키는 공내 수압(breakdown pressure, Pb)과 Kirsch 해법으로 모사되는 시추공 주변 응력 간의 관계는 아래의 식 (2)로 정리된다.
(2) |
공내 수압이 Pb에 도달한 후, 유도된 인장균열을 통해 주입수가 누출되면서 격리 구간 내부의 수압이 급격히 강하한다. 이후 물 주입을 멈추면 시험 구간 내 잔여 수압이 점차 감소하면서 SHmax 방향으로 유도된 인장균열은 균열에 대해 수직한 방향으로 작용하는 응력, 즉 최소수평주응력(Shmin)에 의해 서서히 닫히게 되는데, 균열이 막 닫히는 순간의 균열 내에 존재하는 잔여 수압과 Shmin이 평형을 이루게 되는 압력을 균열폐쇄압력(shut in pressure, Ps)이라 하며 아래 식 (3)의 관계가 성립된다.
(3) |
Shut in시 시간에 따른 점진적인 압력 감쇠 곡선에서 균열 폐쇄 압력의 크기를 결정할 때 주의를 요하기 때문에 다양한 통계적인 방법들이 개발되어 있다(Gronseth and Kry, 1983; Evans et al., 1988; Lee and Haimson, 1989). 그러나 본 연구를 위해 수행한 수압파쇄시험에서는 압력을 응력측정 심도에서 직접 측정하지 않고 지표에서 측정한 압력을 이용하였기 때문에, 초기 수압파쇄시험 결과 해석법으로 많이 사용하는 접선기법(Gronseth and Kry, 1983)을 이용해 균열폐쇄압력을 규명하였다. 수압파쇄시험에서 Shmin의 크기를 직접 규명하는 것과 다르게, SHmax의 크기는 식 (2)와 식 (3)을 결합하여 얻는 아래의 관계식 (4)를 이용해 계산할 수 있다.
(4) |
SHmax 산정을 위한 인장강도는 응력측정 구간 암반에 대한 평균 값을 이용하였다(Koh et al., 2009). 심도별 연직응력(Sv)의 크기는 시험 부지 화강암의 평균 단위중량(Jang et al., 2015)을 고려한 상재하중으로 가정하여 아래 식 (5) 로부터 유추하였다.
(5) |
이렇게 분석한 시추공 수압파쇄 응력측정 결과들을 앞서 규명한 최대수평주응력의 방향과 함께 표 1에 정리하였다. 규명한 최소, 최대수평주응력의 크기는 각각 4.0-11.1 MPa, 5.4-18.1 MPa 범위에서 깊이가 증가함에 따라 증가하는 경향을 보였다. 두 수평주응력의 크기에 대한 연직응력(Sv)의 비로 표현되는 최소, 최대 측압계수(Kmin, Kmax)는 각각 1.2-3.0, 1.7-4.0의 범위를 가지며, 기존 수압파쇄 응력 측정연구 결과들과 비슷하게 깊이가 증가함에 따라 측압계수는 감소하는 추세를 나타냈다(Brown and Hoek, 1978; Sheorey, 1994; Şen and Sadagah, 2002; Bae et al., 2016).
4.2 시추공 공벽파괴 분석
부지 암반의 보다 깊은 심도(~630 m)에 대한 수평응력의 크기는 DB-02 공 영상검층에서 관찰된 DITF와 암반 인장강도 사이의 관계를 이용하여 유추할 수 있었다. 앞서 언급했듯이 시추공 굴착으로 유도되는 벽면 접선응력이 암반 인장강도보다 작아질 때 발생하는 인장파괴는 수평응력의 방향 외에도 크기를 유추하는데 중요한 요소로 활용될 수 있다. DITF는 시추공 굴착 시 이용하는 순환수의 과도한 압력에 의한 자연적인 수압파쇄 형태로 발달하거나, 심부 환경에서 두 수평주응력의 차이가 큰 조건에서 인장파괴로 발생한다. 본 연구의 DB-02 시추에서는 순환수에 진흙을 섞지 않은 청수를 사용했으므로 수압파쇄 조건을 배제하면, 관찰된 DITF는 수평응력편차에 의해 발생한 인장파괴라고 판단할 수 있다. 이러한 가정 아래 DB-02 공 내 DITF가 관찰된 4개 구간에 대하여 주어진 최소수평주응력 및 연직응력조건에서 stress polygon 및 DITF 발생 기준을 이용해 최대수평주응력의 범위를 제한하였다(e.g., Zoback et al., 2003).
그림 8은 뚜렷한 DITF가 관찰된 DB-02 시추공심도 559 m 구간에 대하여 위와 같은 접근방법으로 SHmax의 범위를 유추하는 예를 보여준다. Anderson(1951) 단층 분류체계와 Coulomb 마찰 이론에 근거하여, 현생응력 조건에서 암반 내 존재하는 기존 균열의 미끄러짐이 발생하지 않는 응력의 한계 평형 상태는 식 (6)으로 표현된다.
(6) |

An example of constraining range of SHmax magnitudes at a depth of 559 m in DB-02. The outline of polygon limits possible range of stress state in frictional equilibrium at a depth, under the given Sv and friction condition. If DITF exist, range of stress magnitudes is more constrained in to upper side of DITF criterion, gray shaded area. Under the given conditions of lithostatic Sv, hydrostatic P0, frictional coefficient of 0.6, and Shmin from HF result, the magnitude of SHmax at the DITF depth is constrained to be within 28.4-38.8 MPa.
위에서 S1, S3는 각 암반 응력 체계 아래서 세 주응력(Sv, Shmin, SHmax) 중 가장 크거나 작은 응력 성분이며, m는 균열의 마찰계수이다. 해당 심도에 대해 주어진 Sv와 P0, μ조건 아래서 위 상태를 만족하는 Shmin 및 SHmax의 한계는 polygon의 외곽선으로 그려지며, 두 수평주응력의 범위는 도식화된 polygon 내부로 한정된다. 여기에 벽면 접선응력과 T0의 관계로 정립되는 시추공벽 인장파괴 발생 기준(σθθ ≤ -T0)을 주응력의 항으로 도시할 수 있으며, 초기응력의 범위는 DITF 기준선 위쪽의 보다 좁은 영역으로 제한된다. 이후 DITF 심도에 대해 추정된 Shmin의 크기를 도시하면, 이 선이 DITF 기준 또는 polygon의 외곽선과 교차하는 두 점을 찾게 되는데, 이들은 각각 공벽파괴 또는 한계 평형 조건을 충족하는 SHmax의 최소, 최대 범위를 지시한다. 즉, DB-02 시추공 심도 559 m 구간에 대해서 유추된 SHmax의 범위는 20.4-31.4 MPa 이다. 이 분석에 필요한 매개변수 중 μ 값은 0.6, T0는 DB-02 시추공 암반의 평균 인장강도, 약 10 MPa를 이용하였다(Jang et al., 2015). 이렇게 분석된 심부 응력 규명 결과들을 표 2로 정리하였다. 깊이별 초기 공극압과 연직응력의 크기는 각각 정수압과 상재 하중을 이용해 계산하였으며, 최소수평주응력의 크기는 수압파쇄시험에서 얻어진 심도별 Shmin의 증가 양상을 이용해 유추하였다. DITF 기준으로 제한된 최대수평주응력의 최소 범위는 28.4-37.9 MPa로, 깊이에 따라 수압파쇄로 규명된 천부 SHmax와 비슷한 경향으로 증가하는 양상을 보인다.
5. KURT 부지 암반 응력장
그림 9는 본 연구에서 수행한 시추공 수압파쇄시험 및 공벽파괴 분석을 통해 KURT 부지 천부 및 심부 응력 크기를 분석한 결과들을 보여준다. 응력 측정 결과를 통해 부지 암반은 연직응력이 최소주응력인 역단층성(Sv < Shmin < SHmax) 운동에 유리한 응력 체계(stress regime)하에 있음을 파악할 수 있다. 다만, 깊이에 따라 증가하는 Sv는 약 700 m 이하에서 Shmin을 역전하는 양상이 보이는데, 이는 더 깊은 심부 환경에서는 암반 응력장이 주향이동단층성(Shmin < Sv < SHmax) 응력 체계로 전이됨을 지시한다. Synn et al. (2013)은 최근 30년 이내의 기간 동안 국내에서 수압파쇄 및 응력개방법(overcoring)으로 측정된 460개 응력 자료를 종합하여 깊이에 따른 측압계수의 변화 양상을 정리하였다. 그들의 결과에 따르면 약 800 m 이하 심도에서 최소측압계수(Kmin)가 1보다 작아지는, 즉 Sv가 Shmin의 크기를 역전하는 현상을 파악할 수 있는데, 본 연구의 KURT 부지 응력장 분석 결과 또한 이러한 양상을 잘 반영하고 있다.

Investigated in situ stress state profile as a function of elevation at the KURT site. Both Shmin and SHmax measured by hydraulic fracturing test, tend to increase with increasing test depths. Our stress result indicates that stress regime in the KURT site is in favor of thrust faulting (Sv < Shmin < SHmax). Another noticeable aspect of the stress condition at this site is that Sv is intermediate between two horizontal stresses ~700 m below. It signifies a transition from near surface thrust faulting regime to one favoring strike-slip (Shmin < Sv < SHmax).
본 연구에서 규명된 암반 초기응력 상태를 이용하여, 현생 응력 조건 아래서 KURT 부지 내 불연속면들에 대한 전단성향(slip tendency)을 분석했다. 주어진 응력 조건에서 임의의 방향을 갖는 면에 작용하는 전단응력(τ)과 수직응력(σn)을 계산했고, 해당 면에 대한 전단성향의 크기(τ/σn)를 스테레오넷을 이용하여 각 면의 극점(pole) 위치에 도시하였다(그림 10). 불연속면의 극점의 위치가 붉은색 영역에 가까울수록, 해당 면은 주어진 응력 상태에서 비교적 미끄러지기 쉬운(optimally oriented) 자세를 취하고 있음을 의미한다. Oh et al. (2017)에 따르면, KURT 시설 내부 및 주변 지표지질조사를 통해 파악된 부지 내 불연속면들은 다양한 방향(ubiquitous)으로 분포하는 소규모 균열 및 선구조(joint, foliation)들과 NS 또는 EW, NE-SW 주향의 주요 단층들로 구분된다. 조사된 불연속면들의 극점을 스테레오넷상에 투영한 결과 소규모 구조들은 응력 상태와 관계 없이 임의의 방향으로 분포하는 추세를 보이며, 단층들은 비교적 전단성향이 높은 영역으로 밀집해 나타나는 경향이 관찰되었다. 이는 부지 암반 내 지하수 유동이나 전단 재활성이 주요 단층대를 따라 우선하여 발생할 가능성이 있음을 시사한다(Morris et al., 1996; Moeck et al., 2009; Oh et al., 2010). 다만 재활 가능 측면에서 불연속면들에 대한 역학적 안정성을 분석해보면, 전체적인 전단성향의 크기가 일반적인 균열의 마찰계수 범위(μ=0.6-1.0) 보다 작기 때문에, 부지 내 지질 구조들은 현생 응력 조건 아래서 비교적 안정한 상태에 있는 것으로 판단된다. 그러나, 향후 KURT 부지를 활용한 지하 구조물 기초 설계 시 주어진 초기응력상태, 암반 내 지하수압, 특히 피압(over pressure) 조건 등을 고려해 주 단층들의 역학적 안정성을 면밀히 분석해야 할 필요가 있다.
6. 결 론
본 연구에서는 한국원자력연구원 내 KURT 부지암반에 작용하는 초기응력텐서를 규명하였다. KP-2, YS-04 두 시추공의 수압파쇄시험과 DB-02 시추공의 공벽파괴를 이용해 종합적으로 분석된 암반 초기응력의 방향은 EW의 SHmax 압축방향을 나타낸다. 수압파쇄 결과로부터 천부 암반의 응력장은 역단층성 운동에 유리한 응력 체계하에 있음이 파악되었다. 또한 심부 조건에서는 심도가 깊어짐에 따라 Sv의 크기가 Shmin을 역전하여 암반 응력 체계가 주향이동단층 영역으로 전이되는 경향이 관찰되었다. 이러한 초기응력 분석 결과는 기존에 연구되어온 국내 지역 응력 특성을 잘 반영하고 있다.
규명된 초기응력을 활용하여 KURT 및 부지 주변에 분포하는 불연속면들의 전단성향을 분석한 결과, NS 방향의 주요 단층들은 주어진 응력 조건 아래서 비교적 높은 전단성향을 갖는 자세로 분포함이 확인되었다. 그럼에도 불구하고 KURT 부지에 작용하는 최대전단성향의 크기가 예상되는 균열의 마찰계수범위보다 낮은 값을 보이므로, 현생 응력 조건 아래 불연속면 들은 전반적으로 안정한 상태를 유지할 것이라 판단된다.
본 연구의 결과는 향후 URL 건설 등 부지 활용을 위한 다양한 연구들에 기본적인 설계 변수 획득 방법을 제공할 수 있을 것이라 기대된다. 초기응력 자료만으로 수 년간에 걸친 암반의 응력장 변화와 수리학적, 역학적 거동에 기인한 KURT 부지의 지질역학적 안정성을 총체적으로 분석하지는 못하겠지만, 응력 조건은 설계 초기 또는 직후의 안정성 평가에 기초적인 매개변수로 요구되기 때문이다.
Acknowledgments
본 연구는 미래창조과학부 원자력연구개발사업의 지원(NRF-2017M2A8A5014858)으로 수행되었으며 이에 감사드립니다. 그리고 건설적인 제언으로 이 논문의 질을 더욱 개선할 수 있게 도와주신 두 익명의 심사자께도 감사를 드립니다.
REFERENCES
- Anderson, E.M., (1951), The Dynamics of Faulting, Oliver and Boyd, Edinburgh.
- Bae, S., and Jeon, S., (2005), Characteristics of the Horizontal Stress and the Possibility of Stress Induced Brittle Failure in Chuncheon-Yanggu Mountainous Region by the In-situ Stress Measurements, Tunnel and Underground Space, 15, p157-167, (in Korean with English abstract).
-
Bae, S., Jeon, S., Kim, J., and Park, K., (2016), Study on the Current Horizontal Stress Characteristics of the Tertiary Rock Formations in the Pohang Basin by Integrated Analysis with In-situ Rock Stress Measurement and Borehole Scanning Data Set, Tunnel and Underground Space, 26, p304-315, (in Korean with English abstract).
[https://doi.org/10.7474/tus.2016.26.4.304]
- Bae, S., Jeon, S., and Min, K.B., (2014), In-situ Rock Stress State of Shallow Crustal Region in Korea and Stress Induced Progressive Brittle Failures, ISRM International Symposium - 8th Asian Rock Mechanics Symposium (Proceedings), Sapporo, October 15-17, p2167-2174.
-
Barton, C.A., and Zoback, M.D., (1994), Stress perturbations associated with active faults penetrated by boreholes: Possible evidence for near-complete stress drop and a new technique for stress magnitude measurement, Journal of Geophysical Research: Solid Earth, 99, p9373-9390.
[https://doi.org/10.1029/93jb03359]
- Baumgärtner, J., and Zoback, M.D., (1989), Interpretation of Hydraulic Fracturing Pressure-Time Records Using Interactive Analysis Methods, International Journal of Rock Mechanics and Mining Sciences and Geomechanics Abstracts, 26, p461-469.
-
Brady, P., Arnold, B., Freeze, G., Swift, P., Bauer, S., and Kanney, J., et al. , (2009), Deep borehole disposal of highlevel radioactive waste, Research Report SAND2009-4401, Sandia National Laboratories, CA, US, p74.
[https://doi.org/10.2172/985495]
-
Brown, E.T., and Hoek, E., (1978), Trends in relationships between measured in-situ stresses and depth, International Journal of Rock Mechanics and Mining Sciences and Geomechanics Abstracts, 15, p211-215.
[https://doi.org/10.1016/0148-9062(78)91227-5]
-
Brudy, M., Zoback, M.D., Fuchs, K., Rummel, F., and Baumgärtner, J., (1997), Estimation of the complete stress tensor to 8 km depth in the KTB scientific drill holes: Implications for crustal strength, Journal of Geophysical Research: Solid Earth, 102, p18453-18475.
[https://doi.org/10.1029/96jb02942]
-
Chang, C., and Jo, Y., (2015), Heterogeneous in situ stress magnitudes due to the presence of weak natural discontinuities in granitic rocks, Tectonophysics, 664, p83-97.
[https://doi.org/10.1016/j.tecto.2015.08.044]
-
Chang, C., Jo, Y., Oh, Y., Lee, T.J., and Kim, K.Y., (2014), Hydraulic fracturing in situ stress estimations in a potential geothermal site, Seokmo Island, South Korea, Rock Mechanics and Rock Engineering, 47, p1793-1808.
[https://doi.org/10.1007/s00603-013-0491-7]
- Chang, C., Jo, Y., Quach, N., Shinn, Y.J., Song, I., and Kwon, Y.K., (2016), Geomechanical characterization for the CO2 injection test site, offshore Pohang Basin, SE Korea, 50th US Rock Mechanics/Geomechanics Symposium (Proceedings), Houston, June 26-29, p1064-1069.
-
Chang, C., Lee, J.B., and Kang, T.S., (2010), Interaction between regional stress state and faults: Complementary analysis of borehole in situ stress and earthquake focal mechanism in southeastern Korea, Tectonophysics, 485, p164-177.
[https://doi.org/10.1016/j.tecto.2009.12.012]
- Cho, W.J., Kwon, S., and Choi, J.W., (2008), Thermal Conductivity of Granite from the KAERI Underground Research Tunnel Site, Tunnel and Underground Space, 18, p219-225, (in Korean with English abstract).
-
Evans, K.F., (2005), Permeability creation and damage due to massive fluid injections into granite at 3.5 km at Soultz: 2. Critical stress and fracture strength, Journal of Geophysical Research: Solid Earth, 110, p1-14.
[https://doi.org/10.1029/2004jb003169]
-
Evans, K.F., Scholz, C.H., and Engelder, T., (1988), An analysis of horizontal fracture initiation during hydrofrac stress measurements in granite at North Conway, New Hampshire, Geophysical Journal, 93, p251-264.
[https://doi.org/10.1111/j.1365-246x.1988.tb02000.x]
- Gronseth, J.M., and Kry, P.R., (1983), Instantaneous shut-in pressure and its relationship to the minimum in situ stress, In: Hydraulic Fracturing Stress Measurements, National Academy Press, Washington, DC, p230-257.
-
Haimson, B.C., and Cornet, F.H., (2003), ISRM suggested methods for rock stress estimation-part 3: Hydraulic fracturing (HF) and/or hydraulic testing of pre-existing fractures (HTPF), International Journal of Rock Mechanics and Mining Sciences, 40, p1011-1020.
[https://doi.org/10.1016/j.ijrmms.2003.08.002]
-
Haimson, B.C., Lee, M.Y., and Song, I., (2003), Shallow hydraulic fracturing measurements in Korea support tectonic and seismic indicators of regional stress, International Journal of Rock Mechanics and Mining Sciences, 40, p1243-1256.
[https://doi.org/10.1016/s1365-1609(03)00119-9]
-
Hawkes, C.D., McLellan, P.J., and Bachu, S., (2005), Geomechanical factors affecting geological storage of CO2 in depleted oil and gas reservoirs, Journal of Canadian Petroleum Technology, 44, p52-61.
[https://doi.org/10.2118/05-10-05]
- Heidbach, O., Rajabi, M., Reiter, K., and Ziegler, M., (2016), World Stress Map 2016, GFZ Data Service. Science, 277, p1956-1962.
-
Heidbach, O., Tingay, M., Barth, A., Reinecker, J., Kurfeß, D., and Müller, B., (2010), Global crustal stress pattern based on the World Stress Map database release 2008, Tectonophysics, 482, p3-15.
[https://doi.org/10.1016/j.tecto.2009.07.023]
- Jaeger, J.C., Cook, N.G.W., and Zimmerman, R., (2009), Fundamentals of rock mechanics, Blackwell Publishing, Malden, p475.
-
Jang, H.S., Choe, M.M., Bae, D.S., Kim, G.Y., and Jang, B.A, (2015), Prediction of Brittle Failure within Mesozoic Granite of the Daejeon Region, The Journal of Engineering Geology, 25, p357-368, (in Korean with English abstract).
[https://doi.org/10.9720/kseg.2015.3.357]
- Ji, S.H., Koh, Y.K., Kuhlman, K.L., Lee, M.Y., and Choi, J.W., (2013), Influence of Pressure Change During Hydraulic Tests on Fracture Aperture, Groundwater, 51, p298-304.
-
Jun, M.S., (1991), Body-wave analysis for shallow intraplate earthquakes in the Korean Peninsula and Yellow Sea, Tectonophysics, 192, p345-357.
[https://doi.org/10.1016/0040-1951(91)90108-5]
- Kirsch, G., (1898), Die Theorie der Elastizität und die Bedürfnisse der Festigkeitslehre, Zeitschrift des Vereines deutscher Ingenieure, 42, p797-807.
- Koh, Y.K., Kim, G.Y., Bae, D.S., Kim, K.S., Ryu, J.H., and et al. , (2009), Hydraulic fracturing test in the YS-04 borehole, Research Report KAERI/TR-3843/2009, Korea Atomic Energy Research Institute, Korea, p57, (in Korea with English abstract).
- Kwon, S., and Cho, W.J., (2007), Rock Mechanics Studies at the KAERI Underground Research Tunnel for High-Level Radioactive Waste Disposal, Tunnel and Underground Space, 17, p43-55, (in Korea with English abstract).
-
Kwon, S., Cho, W.J., and Choi, J.W., (2011), Initial thermal conditions around an underground research tunnel at shallow depth, International Journal of Rock Mechanics and Mining Sciences, 48, p86-94.
[https://doi.org/10.1016/j.ijrmms.2010.08.011]
- Lee, C., Kwon, S., Choi, J. won, and Jeon, S., (2011), An Estimation of the Excavation Damaged Zone at the KAERI Underground Research Tunnel, Tunnel and Underground Space, 21, p359-369, (in Korea with English abstract).
-
Lee, M.Y., and Haimson, B.C., (1989), Statistical Evaluation of Hydraulic Fracturing Stress Measurement Parameters, International Journal of Rock Mechanics and Mining Sciences, 26, p447-456.
[https://doi.org/10.1016/0148-9062(89)91420-4]
-
Martin, C.D., and Lanyon, G.W., (2003), Measurement of in-situ stress in weak rocks at Mont Terri Rock Laboratory, Switzerland, International Journal of Rock Mechanics and Mining Sciences, 40, p1077-1088.
[https://doi.org/10.1016/s1365-1609(03)00113-8]
-
Moeck, I., Kwiatek, G., and Zimmermann, G., (2009), Slip tendency analysis, fault reactivation potential and induced seismicity in a deep geothermal reservoir, Journal of Structural Geology, 31, p1174-1182.
[https://doi.org/10.1016/j.jsg.2009.06.012]
-
Morris, A., Ferrill, D.A., and Henderson, D.B., (1996), Slip-tendency analysis and fault reactivation, Geology, 24, p275-278.
[https://doi.org/10.1130/0091-7613(1996)024<0275:staafr>2.3.co;2]
- Oh, C.W., Yengkhom, K.S., Yi, S., Lee, S., and Lee, B., (2017), The study on the tectonic evolution of the Yuseong area, Research Report KAERI/CM-2379/2016, Korea Atomic Energy Research Institute, Korea, p90, (in Korean with English abstract).
- Oh, Y., Chang, C., and Lee, T.J., (2010), Distinguishing permeable fractures in crystalline rock using well log data: case study in Seokmo Island, Journal of the Geological Society of Korea, 46, p585-607, (in Korean with English abstract).
- Park, K.W., Koh, Y.K., Kim, K.S., and Kim, K.Y., (2010), Fracture zones in deep borehole in KURT, Research Report KAERI/TR-4010/2010, Korea Atomic Energy Research Institute, Korea, p52, (in Korean with English abstract).
-
Schmitt, D.R., Currie, C.A., and Zhang, L., (2012), Crustal stress determination from boreholes and rock cores: Fundamental principles, Tectonophysics, 580, p1-26.
[https://doi.org/10.1016/j.tecto.2012.08.029]
-
Scholz, C.H., (2002), The mechanics of earthquakes and faulting, Cambridge University Press, Cambridge, p473.
[https://doi.org/10.1017/cbo9780511818516]
- Şen, Z., and Sadagah, B.H, (2002), Probabilistic horizontal stress ratios in rock, Mathematical Geology, 34, p845-855.
-
Sheorey, P.R., (1994), A Theory for in-Situ Stresses in Isotropic and Transversely Isotropic Rock, International Journal of Rock Mechanics and Mining Sciences Geomechanics Abstracts, 31, p23-34.
[https://doi.org/10.1016/0148-9062(94)92312-4]
-
Stephansson, O., and Zang, A., (2012), ISRM suggested methods for rock stress estimation-part 5: Establishing a model for the in situ stress at a given site, Rock Mechanics and Rock Engineering, 45, p955-969.
[https://doi.org/10.1007/s00603-012-0270-x]
-
Synn, J.H., Park, C., and Lee, B.J., (2013), Regional Distribution Pattern and Geo-historical Transition of In-situ Stress Fields in the Korean Peninsula, Tunnel and Underground Space, 23, p457-469, (in Korean with English abstract).
[https://doi.org/10.7474/tus.2013.23.6.457]
- Yale, D.P., (2003), Fault and stress magnitude controls on variations in the orientation of in situ stress, In: Ameen, M (ed.), Fracture and In-Situ Stress Characterization of Hydro Carbon Reservoirs, Geological Society, London, Special Publication, 209, p55-64.
-
Yoon, C.H., Choi, Y.C., Kwon, S., and Choi, H.J., (2013), A Study on the Temperature Distribution of Rock Mass at KAERI Underground Research Tunnel: Verification on the Result of Borehole Heater Test, Tunnel and Underground Space, 23, p297-307, (in Korean with English abstract).
[https://doi.org/10.7474/tus.2013.23.4.297]
-
Zoback, M.D., Apel, R., Baumgärtner, J., Brudy, M., Emmermann, R., and et al. , (1993), Upper-crustal strength inferred from stress measurements to 6 km depth in the KTB borehole, Nature, 365, p633-635.
[https://doi.org/10.1038/365633a0]
-
Zoback, M.D., Barton, C.A., Brudy, M., Castillo, D.A., Finkbeiner, T., and et al. , (2003), Determination of stress orientation and magnitude in deep wells, International Journal of Rock Mechanics and Mining Sciences, 40, p1049-1076.
[https://doi.org/10.1016/j.ijrmms.2003.07.001]
-
Zoback, M.D., and Harjes, H.-P., (1997), Injection-induced earthquakes and crustal stress at 9 km depth at the KTB deep drilling site, Germany, Journal of Geophysical Research: Solid Earth, 102, p18477-18492.
[https://doi.org/10.1029/96jb02814]